1.IntroductionA major problem in cancer chemotherapy is still resistance, namely the lack of responsivity that tumor cells display toward chemotherapeutic drugs. In the case of multidrug resistance (MDR), a well-characterized form of resistance, tumor cells exposed to a single drug become resistant to drugs and molecules of unrelated chemical structures such as anthracyclines, taxols, vinka alkaloids, and a few fluorescent dyes. As widely reviewed in the literature,1 2 3 4 MDR is a complex multifactorial phenomenon, correlated with different phenotypic alterations, such as the alkalization of the intracellular pH 5 6 and an expanded secretory network.7 MDR cells overexpress one or more glycoproteins, which are responsible for an enhanced cellular detoxification. Among these glycoproteins the 170 kD P-glycoprotein (P-gp 170), encoded by the mdr1 gene, has been widely characterized.8 9 10 It has been suggested that this protein is an ATP-ase that pumps drug molecules out of the cell, reducing therefore the intracellular drug accumulation. Other overexpressed proteins are the 190 kD multidrug resistance associated protein (MRP 1),11 12 which participates to cellular detoxification through the glutathione pathway,13 and the lung resistance protein (LRP), which is probably involved in the outward transport of drugs from the nucleus.3 In spite of the large number of studies reported in the last decade,14 15 the understanding of drug resistance is still incomplete. Little is known about the interactions and the intracellular localization of drugs in MDR cells,3 16 17 18 19 and about the cell biology of resistant and MDR cells.4 20 21 22 Recently, it has been suggested that the resistance of tumor cells to anticancer drugs is related to the cell capacity of escaping apoptosis.23 Indeed, in addition to its role in drug efflux, P-gp 170 has been shown to protect resistant cells from apoptosis and in particular from caspase-dependent apoptosis.24 25 26 New chemotherapeutic agents are therefore under study to specifically overcome the blocks in the apoptosis pathway of resistant cells.27 28 29 In this perspective, attention has been devoted to mitochondria due to their critical role in apoptosis.30 31 32 The study of mitochondria in tumor cells is therefore an important subject for the understanding of resistance. To this end, we have undertaken a laser scanning confocal fluorescence microscopy (LSCFM) study in single living cells of rhodamine 123 (R123), a potentiometric vital probe of mitochondria, whose specificity is well documented in the literature.23 33 34 We present here the R123 study in the human carcinoma MCF-7 cell line, a good model system for the investigation of mitochondria in tumor cells,35 due to the high uptake of R123 that characterizes this cell line also in presence of multidrug resistance. The results obtained for sensitive MCF-7 and for multidrug resistant MCF-7/DX cells indicate that mitochondria are different for localization, morphology, and activity in the two cell lines. In addition it has been found that in MDR cells a drastic rearrangement in the system of active mitochondria follows the treatment with verapamil, a calcium channel blocker that is known to revert MDR.36 37 After this treatment, the pattern of mitochondria seen in sensitive cells is observed in the “reverted” MDR cells. We also present here the study of R123 in human lung carcinoma A549, a cell line known to overexpress the resistance protein LRP and to be highly unaffected by antitumor drugs.38 We have found that mitochondria in this cell line share interesting features with those of multidrug resistant carcinoma MCF-7/DX cells. To obtain these results, we have taken advantage of the high sensitivity of the photon counting detection of the fluorescence confocal microscope employed in this study (MRC-600, Bio-Rad). This detection has enabled us to image—at the highest spatial resolution allowed by confocal optics—mitochondria in their functional state, by reducing the laser power excitation to values that do not damage cell viability and functions,17 19 a crucial condition for the study of mitochondria in living cells.39 Furthermore, by image analysis we have characterized the morphology of peripheral mitochondria, newly observed in resistant cells. 2.Materials and Methods2.1.ChemicalsR123, dimethylaminostyryl-methylpyridiniumiodine (DASPMI), C 6–NBD –ceramide and verapamil were purchased from Molecular Probes (Eugene, OR), doxorubicin from Pharmacia-Farmitalia, Nerviano, Italy. The stock solution concentrations of R123 and DASPMI were determined by spectrophotometric methods. 2.2.Cell CultureThe human breast carcinoma MCF-7 cell line and its derived multidrug resistant subline MCF-7/DX were a kind gift of Professor M. Manfait (Reims University, Reims, France). To confirm the data obtained on these cell lines, we repeated the experiments on the sensitive and resistant MCF-7 cell lines given by Dr. G. Arancia (Istituto Superiore di Sanita`, Roma, Italy), who also kindly provided the human lung carcinoma A549, a cell line that is resistant to doxorubicin. The MCF-7/DX and A549 cell lines are known to overexpress, respectively, the Pgp-170 glycoprotein40 and the LRP.38 Other carcinoma cells were also examined: the human ovary adenocarcinoma A2780 cell line and its multidrug resistant sublines A2780/706, A2780/388, A2780/1039, the human colon adenocarcinoma LoVo cell line and its MDR subline LoVo/DX, all kindly given by Dr. Dario Ballinari (Pharmacia, Milan, Italy). The MCF-7, A549, A2780 cells and their MDR sublines were grown as monolayers in RPMI 1640 (Hyclone, Cramlington, UK) supplemented with 10 FCS (Hyclone), 2 mM L-glutamine, and antibiotics at 37 °C, 5 CO 2. LoVo and LoVo/DX cells were grown in Ham’s F12K medium with 10 FCS (Hyclone), 2 mM L-glutamine, and antibiotics at 37 °C, 5 CO 2. The resistant MCF-7/DX cells were cultured in the presence of 1 μM doxorubicin, while the LoVo/DX cells were maintained in the presence of 170 nM doxorubicin. Before the use, resistant cells were grown for at least 2–4 weeks in drug-free medium. 2.3.Sample PreparationThe cells were seeded in 35 mm Petri dishes and allowed to grow, at 37 °C and 5 CO 2, up to 50–70 confluence. At this stage the medium was removed and the cells were washed twice with phosphate buffered saline (PBS) and incubated at 37 °C and 5 CO 2 in the growth medium containing the appropriate dye or drug for each specific experiment. For R123 staining, cells were incubated for 10 min in 1 μM R123 solution. This staining procedure has been optimized in order to allow cell viability and a high R123 accumulation. This last requirement is essential to study mitochondria in the MDR sublines, as R123 has been shown to be a substrate for outward transport in MDR cells. In the case of DASPMI, a concentration of 10μM was used with an incubation time of 30 min. After incubation, cells were washed twice with PBS. Few microliters of PBS were added before the coverslip was placed on to prevent cell drying. For the vital staining of the Golgi apparatus41 50 nmol of C 6–NBD –ceramide were dissolved in 10 mL of Hepes-buffered minimal essential medium (HMEM) containing 0.34 mg/mL of defatted BSA. The solution was dialyzed for 12 h at 4 °C against 500 mL of HMEM. A final solution of the complex C 6–NBD –ceramide/BSA at a concentration of 5 μM was used for a 30 min incubation of MCF-7 cells. Cells were then washed twice before measurements. For the experiment with FCCP, cells were incubated with 1 μM R123 for 10 min as described earlier. After washing, 1 mL of PBS was added to the Petri dish. A LSCFM image was collected by the Nikon 20× air objective [numerical aperature (NA)=0.4] . Then 50 μL of the FCCP stock solution was added, to reach the final concentration of 100 μM.42 Cells were again imaged 10 min after the addition of FCCP, in the same optical conditions. For the verapamil experiment on MCF-7/DX cells, cells were preincubated in culture medium with 100 μM verapamil for 10 min at 37 °C, 5 CO 2. Then a small volume of a R123 solution was added to reach the final concentration of 1 μM. After 10 min of this double incubation in the presence of R123 and verapamil, cells were washed with PBS. A few microliters of a 100 μM verapamil solution were then added to the cells before the coverslip was placed on. The whole procedure was completed in about 30 min. The R123 fluorescence images were then collected immediately after. For the experiment on MCF-7/DX cells after a short verapamil treatment, cells were incubated in a 100 μM verapamil solution for 10 min as before. Then a R123 solution was added to the cells at a final concentration of 1 μM, removing immediately the medium in excess without any washing, in order to inspect immediately the cells. The coverslip was placed on the cells and the measurement was started about 12 min after the addition of verapamil. 2.4.Confocal Fluorescence MicroscopyThe intracellular distribution of R123, DASPMI, and C 6–NBD –ceramide were studied by laser scanning confocal microscopy using the MRC-600 microscope (Bio-Rad, Hemel Hempstead, UK). The scanning head of the MRC-600 has been coupled to an upright epifluorescence microscope Nikon Optiphot-2 (Nikon, Tokyo, Japan) with the oil immersion objective Nikon Planapochromat 60× (NA 1.4). A 20× objective was instead employed when a larger field of view was required. A 25 mW argon laser was used for fluorescence excitation at 488 nm. The fluorescence emission was detected, through a long pass filter above 515 nm, by the fast photon counting mode of the MRC-600 microscope. The high sensitivity of the photon counting detection has allowed us to employ a low laser power excitation of 0.1 mW at the entry of the optical head. This is a crucial condition for the study of active mitochondria in living cells, since mitochondria are known to be damaged by light.39 43 In particular, we have observed that peripheral mitochondria can be seriously damaged by continuous irradiation. For their detection it has been therefore essential to employ a scanning excitation at low power. The R123 distribution in the cell lines MCF-7, MCF-7/DX, and A549 was found to be fully reproducible under condition of cell viability (over 500 cells examined in 10 independent experiments for the MCF-7 cell lines). 2.5.Image AnalysisIn order to see the morphology of the two different pools of mitochondria, image processing has been performed by using the LaserPix program from Bio-Rad (Hemel Hempstead, UK). Two morphological filters have been used: the white top hat filter and the black top hat filter. The white top hat filter emphasizes the brighter structures and, by enhancing the contrast it makes possible to see the organelle shape. The black top hat displays only the structures contour, setting to black the fluorescent core of the structure itself. The effect of this latter filter can be well appreciated when a gray scale inversion is applied. In this work we have used the following filters setting: Kernel size=7×7; passes=1; strength=10. 3.Results3.1.Different Mitochondria in Sensitive and Multidrug Resistant CellsThe intracellular distribution of rhodamine 123 has been studied by LSCFM in sensitive and multidrug resistant MCF-7 carcinoma cells. In sensitive cells [Fig. 1(a)], highly fluorescent mitochondria are observed in the cytoplasm with the exclusion of the Golgi region as described in the literature.33 34 43 To better visualize this localization we have studied in MCF-7 cells the distribution of C 6–NBD –ceramide [see Fig. 1(b)], a vital stain of the Golgi system. By comparing Figs. 1(a) and 1(b), it is possible to see that mitochondria are crowded around the nucleus and spread toward the cell periphery. They display polymorphic structures, with spherical, tubular, and bean-like shapes.35 44 45 This morphology will be better appreciated after the image analysis reported in the next paragraph. Figure 1Fluorescence images of sensitive human breast carcinoma MCF-7 cells: (a) active mitochondria stained by rhodamine 123 and (b) the Golgi apparatus stained by the vital fluorescent probe C 6–NBD -ceramide. ![]() In multidrug resistant MCF-7/DX cells, instead, an unexpected result is found (Fig. 2). In these cells no active mitochondria are observed in the central cytoplasm, but only in the subplasmalemmal region, where they constantly display a “punctate” pattern. As will be shown by the image analysis in the next paragraph, these peripheral mitochondria exhibit a peculiar morphology with a star-lobed organization. The overall R123 fluorescence intensity of these mitochondria in MCF-7/DX cells is reduced by a factor of 2 compared with that of mitochondria in sensitive cells. This is an expected result since R123 has been shown to be a substrate for the enhanced MDR efflux mechanism.16 18 We should also note that MCF-7/DX cells have a different morphology compared with sensitive cells, forming looser colonies and displaying several filopodia. In the next section we will discuss the possible meaning of these morphological characteristics. The differences in the mitochondria of MCF-7 and MCF-7/DX cells have been confirmed by a different potentiometric probe, DASPMI. Again active mitochondria are observed through the whole cytoplasm in sensitive cells [Fig. 3(a)], whereas in resistant cells they are confined in the cell periphery [Fig. 3(b)]. Figure 3Fluorescence images of DASPMI, a potentiometric probe of mitochondria, (a) in human breast carcinoma sensitive MCF-7 cells, and (b) in multidrug resistant MCF-7/DX cells. ![]() We have verified the effect of a proton ionophore on R123 fluorescence in MCF-7 and MCF-7/DX cells. Since potentiometric probes are accumulated and retained by the mitochondria membrane potential, no mitochondria fluorescence is expected when the potential is dissipated. Indeed, no R123 fluorescence was detected in both cell lines after the treatment with the uncoupler FCCP (data not shown). This experiment confirms that the two potentiometric probes stain active mitochondria in sensitive and MDR cells. We have also studied the intracellular fluorescence of R123 in human colon adenocarcinoma LoVo cells and in human ovarian carcinoma A2780 cells, as well as in their MDR sublines. In these two sensitive cell lines, active mitochondria were found in the central region of the cytoplasm, displaying the morphology seen in sensitive MCF-7 cells (data not shown). None of these cells showed punctate mitochondria in the subplasmalemmal region. In the LoVo and A2780 MDR sublines, no R123 intracellular fluorescence was instead observed, a result that might be due to a very efficient R123 efflux in these MDR carcinoma cells. Apparently, MCF-7/DX cells have, also in presence of MDR, a high R123 retention that could explain the staining of peripheral mitochondria, not observed under the same conditions in the LoVo and A2780 MDR cell lines. In order to overcome this difficulty we have examined resistant cells not expressing Pgp-170. We have, therefore, studied the R123 fluorescence in human lung carcinoma A549 cells. These cells, which display a low responsivity to doxorubicin,38 are characterized by the overexpression of the LRP. As can be seen in Fig. 4, they display highly fluorescent mitochondria in the perinuclear region of the cytoplasm. These mitochondria are similar for localization and morphology to those observed in sensitive MCF-7 cells [Fig. 1(a)]. In addition, a system of mitochondria can be seen in the cell periphery (Fig. 4), with an average fluorescence intensity 4–5 times weaker than that of central mitochondria. Also in this case, to visualize the morphology of these peripheral mitochondria, we have performed the image analysis that is reported in the next paragraph. Figure 4(a) Fluorescence image of rhodamine 123 in human lung carcinoma A549 cells. In addition to highly fluorescent central mitochondria, mitochondria with a lower fluorescence are also present in the periphery of the cell. (b) Enlarged image of peripheral mitochondria. (c) The fluorescence image b has been processed by the black top hat filter (LaserPix software, Bio-Rad). (d) The processed image c after gray scale inversion. ![]() 3.2.Morphology of Central and Peripheral MitochondriaIn order to compare the morphology of the central and peripheral mitochondria, image analysis procedures have been applied to the confocal R123 fluorescent images of MCF-7 and A549 cells (Figs. 1, 2, and 4). The morphological filters white top hat and black top hat (LaserPix, Bio-Rad) have been used for this study. A high magnification image of a sensitive MCF-7 cell and its image analysis are shown in Fig. 5. The well-known filamentous, bean-shaped and polymorphic morphology of mitochondria33 34 45 can be easily identified by the direct inspection of the fluorescent data [Fig. 5(a)], but it is better appreciated after filter treatments [Figs. 5(c)–5(e)]. Figure 5(a) Fluorescence image of rhodamine 123 in a single sensitive MCF-7 cell. (b) Enlarged view of central mitochondria. Image analysis of b is performed: in (c) by the white top hat filter and in (d) by the black top hat filter (LaserPix software, Bio-Rad); (e) same image as in d after gray scale inversion. ![]() In Figs. 6(a) and 6(b) the apical region of MCF-7/DX cells is reported. Here the morphology of the less fluorescent peripheral mitochondria is significantly improved by image filtering [Figs. 6(c)–6(e)]. This image analysis shows a star-lobed organization not yet reported for mitochondria in living cells. Figure 6(a) Confocal fluorescence image of rhodamine 123 in multidrug resistant MCF-7/DX cells. (b) An enlarged view of peripheral mitochondria. Image analysis of B is performed: in (c) by the white top hat filter (LaserPix software, Bio-Rad) and in (d) by the black top hat filter; (e) same image as in d after gray scale inversion. ![]() The same analysis has been performed on the R123 fluorescence image of an A549 cell reported in Fig. 4. Here the morphology of peripheral mitochondria is hardly appreciated by a visual inspection of the data in Fig. 4, due to their low fluorescence intensity. The application of the black top hat filter allows to recognize a star-lobed organization also in this case. Surprisingly, it is identical to that found for peripheral mitochondria in resistant MCF-7/DX cells. 3.3.Two Populations of Mitochondria Coexist in MCF-7/DX CellsTo explore what happens to the peripheral mitochondria when an MDR revertant is supplied to MCF-7/DX cells, we have studied the effect of verapamil, a well-known revertant of multidrug resistance. As can be seen from Fig. 7, when resistant MCF-7/DX cells are incubated with R123 in the presence of verapamil, a drastic change in the fluorescence distribution is observed: peripheral mitochondria disappear and a pool of mitochondria similar to that of sensitive cells becomes fluorescent, with an intensity at the level of sensitive cells. Figure 7Rhodamine 123 fluorescence in multidrug resistant MCF-7/DX cells after verapamil incubation. This treatment induces a drastic change in the system of active mitochondria: (a) in the adhesion plane peripheral mitochondria are absent; only central mitochondria are seen through the cytoplasm, as in sensitive MCF-7 cells; (b) peripheral subplasmalemmal mitochondria are absent also in the apical plane. ![]() This result could be explained in two alternative ways. One possibility is that verapamil might induce a dislocation of peripheral mitochondria toward the center of the cell and a change in their morphology. Alternatively, two different populations of mitochondria could coexist in the cell. If this is the case, central mitochondria might be present in resistant cells, although not active. To discriminate between these two cases, R123 fluorescence distribution has been studied in resistant cells after a short treatment with verapamil, as explained in Sec. 2. A series of z-section images has been collected in order to explore the peripheral and central regions of the cytoplasm, where the two types of mitochondria are expected to be found. As can be seen in Fig. 8, at the initial stage of the experiment, after 12 min of verapamil incubation, both peripheral and central mitochondria are simultaneously fluorescent. Peripheral mitochondria are localized in the apical region [Fig. 8(a)] while central mitochondria are evident in the adhesion plane 4 μm below [Fig. 8(b)]. At longer times (about 45 min after the addition of verapamil and 30 min after the addition of R123) the fluorescence of peripheral mitochondria disappears and only central mitochondria remain fluorescent as already observed in the experiment of Fig. 7. In a control experiment performed in absence of verapamil but under the same R123 incubation conditions (30 min), only peripheral mitochondria were observed. This excludes that the staining of central mitochondria could be due to a longer R123 incubation time. Figure 8Rhodamine 123 fluorescence in multidrug resistant MCF-7/DX cells after a short treatment with verapamil (10 min). Central and peripheral mitochondria are simultaneously active: (a) peripheral mitochondria are observed in the apical plane; (b) both central and peripheral mitochondria can be seen in the adhesion plane. ![]() This experiment, therefore, seems to indicate that two populations of mitochondria are simultaneously present in multidrug resistant MCF-7/DX cells: a peripheral population of active mitochondria and a central population of mitochondria with no membrane potential, not detectable by potentiometric probes. In these cells verapamil seems to induce a dissipation of membrane potential in peripheral mitochondria, and to reset the potential of central mitochondria to the level of sensitive cells. 4.DiscussionIn this study we have shown that active mitochondria in sensitive and multidrug resistant MCF-7 carcinoma cells are surprisingly different in localization and morphology. We have found that in sensitive MCF-7 cells active mitochondria invade the whole cytoplasm from the perinuclear region to the cell periphery and display a polymorphic morphology as described in the literature.39 46 47 In multidrug resistant cells active mitochondria are instead localized in the subplasmalemmal region, constantly displaying a homogeneous pattern of star-lobed structures (Fig. 6). No mitochondria are instead observed in the inner cytoplasm of multidrug resistant MCF-7/DX. Since R123 is a MDR substrate, the lack of R123 fluorescence on central mitochondria in MCF-7/DX cells might be simply due to the efficient outward Pgp-170 transport which is active in MDR cells. In this hypothesis, Pgp-170 activity would lower the intracellular R123 concentration, particularly in the perinuclear region, therefore limiting the staining of mitochondria. However, in MCF-7/DX cells, as indicated by Figs. 7 and 8, central mitochondria reach the vicinity of the subplasmalemmal region and are contiguous to the fluorescent peripheral mitochondria: they have, consequently, access to the dye. The lack of R123 fluorescence on central mitochondria in these cells seems, therefore, to reflect the absence of membrane potential. Moreover, we have found that the MDR revertant verapamil is able to switch the membrane potential of the two pools of mitochondria, leading to the deenergization of peripheral mitochondria and to the activation of mitochondria in the bulk cytoplasm of MCF-7/DX cells. Since these two pools of mitochondria are alternatively active, they appear to be independent and unconnected. Our results seem, therefore, to suggest that two populations of mitochondria are simultaneously present in resistant MCF-7/DX cells: peripheral mitochondria, which are found to be active and central mitochondria with no membrane potential. Furthermore, we have also shown that the presence of a mitochondria population in the cell periphery is not limited to multidrug resistant MCF-7/DX cells expressing Pgp-170. Indeed, we have observed peripheral mitochondria with the same peculiar morphology also in human lung carcinoma A549 cells, a cell line with a low responsivity to doxorubicin that overexpresses LRP instead of Pgp-170. A question then arises on the functional role of the two mitochondria populations in resistant carcinoma cells. In these last years it has become clear that mitochondria play an important role in cell calcium homeostasis48 and apoptosis.30 31 32 As extensively observed in various cell types,49 50 mitochondria are often found close to the endoplasmic reticulum (ER), i.e., close to the main intracellular calcium store, where they participate to the calcium signaling from the ER.51 However, by electron microscopy Lawrie et al.;52 have shown that in excitable endothelial ECV-304 cells a consistent group of mitochondria is distributed near the plasma membrane. These authors have suggested that mitochondria cluster in this peripheral localization to buffer the high Ca 2+ concentration created by the calcium influx that characterizes excitable cells. In living pancreatic acinar cells53 three populations of mitochondria have been seen to participate independently to the local energy supply and Ca 2+ sequestration, each population being active in a different cell compartment, where they have different functional roles. Near the plasma membrane, for example, peripheral mitochondria are involved in the store-operated calcium entry, while mitochondria in the perigranular region supply ATP for secretion. In immature retinal oligodendrocytes a LSCFM study of rhodamine 12354 has interestingly shown that active mitochondria are confined only to the tips of the cellular processes. However, when these cells were observed by electron microscopy, i.e., by a technique that detects mitochondria independently of their membrane potential, mitochondria were found evenly distributed through the cytoplasm. These results indicate that in these immature cells, similarly to what is found in our MCF-7/DX cells, two mitochondria populations are present: an active population at the cell periphery, and a population with no membrane potential in the central perinuclear region. In this case, it was suggested that the peripheral concentration of active mitochondria might be necessary for the energy supply required by the rapid growing of the processes and for Ca 2+ signaling. These earlier investigations all clearly illustrate that the localization of mitochondria is set to meet the different functional needs of the cell. In particular, the role of peripheral mitochondria is to accumulate calcium at the mouth of plasma membrane Ca 2+ channels and to supply energy for cell motility and secretion. Central mitochondria are instead involved in calcium signaling from the ER and in the cell apoptotic pathways, as recently discussed in the literature.31 32 49 In this perspective, we suggest that the two populations of mitochondria in multidrug resistant MCF-7/DX carcinoma cells have different roles. Peripheral mitochondria might supply ATP for cell detoxification and motility. Indeed a higher motility and invasivity generally characterizes multidrug resistant carcinoma cells compared with their parental sensitive cells.55 In addition, our resistant MCF-7/DX cells display the characteristic morphology of the invasive phenotype, namely a fusiform shape and filopodia.56 57 Interestingly, it has been recently shown by De Larco et al.;58 that doxorubicin, even after a short treatment, permanently increases the invasive potential of MCF-7 cells. After this treatment, sensitive cells undergo an epithelial-to-fibroblastic transition. They acquire a fusiform morphology and the ability to migrate and induce metastasis, characteristic features of tumor progression. Since our multidrug resistant MCF-7/DX cells were grown in presence of doxorubicin, it is possible that also in our case the exposition to the drug was responsible for inducing the metastatic phenotype. In this context, it is interesting to point out that verapamil, which we have found to deactivate peripheral mitochondria in MCF-7/DX cells, is known to inhibit tumor invasion and metastasis.59 Verapamil has been also seen to block cell migration in epidermal dendritic cells expressing Pgp-170.60 Peripheral mitochondria, therefore, seem to have a role in the motility and invasivity of carcinoma cells. We have also reported here the presence of peripheral mitochondria with low membrane potential in lung carcinoma A549, a cell line that is normally noninvasive, but that can undergo a metastatic transformation when exposed to genetic and metabolic stresses.56 57 We suggest that the presence of peripheral mitochondria with low activity in these cells might be an indication of their capacity to become invasive and metastatic. Concerning central mitochondria, we have found that they are active in sensitive cells, but not in multidrug resistant MCF-7/DX cells. This selective deactivation might reflect an alteration of ER calcium signaling as recently seen by Collins et al.;61 in HeLa cells. In human hepatocarcinoma HepG2 cells, Ca 2+ signals from ER to central mitochondria have been shown to trigger apoptosis in presence of proper apoptotic agents.62 The deactivation of central mitochondria in MCF-7/DX cells could, therefore, interfere with the cell apoptotic pathways and contribute to the cell drug resistance. Our suggestion is consistent with the recent finding that tumor cell resistance is often due to blocks in the cell apoptotic pathways.23 25 26 It should also be mentioned that the overexpression of Pgp-170 directly protects resistant tumor cells from caspase-dependent apoptosis,24 25 26 an apoptotic pathway which can be activated by anticancer drugs. The protective role of Pgp-170 against apoptotic death has been suggested to occur also in other cellular systems known to express Pgp, such as stem cells and immune cells.26 63 In these cells, the apoptotic agents might be removed by the efflux activity of Pgp-170.26 Taking into account all these results, we suggest that in multidrug resistant MCF-7/DX cells the lack of activity of central mitochondria and the antiapoptotic action of Pgp-170 might both contribute to drug resistance by blocking the cell apoptotic pathways. The possible correlation between these two protective mechanisms remains at present an open question. The two populations of mitochondria that we have found in resistant MCF7/DX cells with different energetic state, morphology, and localization, seem therefore to be involved in different cellular processes. Further studies of the functional roles of mitochondria in carcinoma cells might then be useful for a better understanding of resistance and tumor progression. They might also help to find new strategies in cancer therapy and detection. AcknowledgmentsThe authors thank G. Arancia and D. Ballinari for kindly supplying the cell lines. They also thank M. Manfait and S. Ottolenghi for helpful discussions. REFERENCES
J. A. Endicott
and
V. Ling
,
“The biochemistry of P-glycoprotein-mediated multidrug resistance,”
Annu. Rev. Biochem. , 58 137
–171
(1989). Google Scholar
J. M. Ford
and
W. N. Hait
,
“Pharmacology of drugs that alter multidrug resistance in cancer,”
Pharmacol. Rev. , 42 155
–199
(1990). Google Scholar
A. K. Larsen
,
A. E. Escargueil
, and
A. Skladanowski
,
“Resistance mechanisms associated with altered intracellular distribution of anticancer agents,”
Pharmacol. Ther. , 85 217
–229
(2000). Google Scholar
M. M. Gottesman
,
T. Fojo
, and
S. E. Bates
,
“Multidrug resistance in cancer: role of ATP-dependent transporters,”
Nat. Rev. Cancer , 2 48
–58
(2002). Google Scholar
P. D. Roepe
,
L. Y. Wei
,
J. Cruz
, and
D. Carlson
,
“Lower electrical membrane potential and altered pHi homeostasis in multidrug-resistant (MDR) cells: further characterization of a series of MDR cell lines expressing different levels of P-glycoprotein,”
Biochemistry , 32 11042
–11056
(1993). Google Scholar
S. Simon
,
D. Roy
, and
M. Schindler
,
“Intracellular pH and the control of multidrug resistance,”
Proc. Natl. Acad. Sci. U.S.A. , 91 1128
–1132
(1994). Google Scholar
R. Martinez-Zaguilan
,
N. Raghunand
,
R. M. Lynch
,
W. Bellamy
,
G. M. Martinez
,
B. Rojas
,
D. Smith
,
W. S. Dalton
, and
R. J. Gillies
,
“pH and drug resistance. I. Functional expression of plasmalemmal V-type
H+-ATPase
in drug-resistant human breast carcinoma cell lines,”
Biochem. Pharmacol. , 57 1037
–1046
(1999). Google Scholar
R. L. Juliano
and
V. Ling
,
“A surface glycoprotein modulating drug permeability in Chinese hamster ovary cell mutants,”
Biochim. Biophys. Acta , 455 152
–162
(1976). Google Scholar
J. H. Gerlach
,
J. A. Endicott
,
P. F. Juranka
,
G. Henderson
,
F. Sarangi
,
K. L. Deuchars
, and
V. Ling
,
“Homology between P-glycoprotein and a bacterial haemolysin transport protein suggests a model for multidrug resistance,”
Nature (London) , 324 485
–489
(1986). Google Scholar
K. Ueda
,
C. Cardarelli
,
M. M. Gottesman
, and
I. Pastan
,
“Expression of a full-length cDNA for the human ‘MDR1’ gene confers resistance to colchicine, doxorubicin, and vinblastine,”
Proc. Natl. Acad. Sci. U.S.A. , 84 3004
–3008
(1987). Google Scholar
P. Borst
,
R. Evers
,
M. Kool
, and
J. Wijnholds
,
“A family of drug transporters: the multidrug resistance-associated proteins,”
J. Natl. Cancer Inst. , 92 1295
–1302
(2000). Google Scholar
D. R. Hipfner
,
R. J. Deeley
, and
S. P. C. Cole
,
“Structural, mechanistic and clinical aspects of MRP1,”
Biochim. Biophys. Acta , 1461 359
–376
(1999). Google Scholar
Z. Benderra
,
A. Trussardi
,
H. Mojani
,
A. M. Villa
,
S. M. Doglia
, and
M. Manfait
,
“Regulation of cellular glutathione modulates nuclear accumulation of daunorubicin in human MCF7 cells overexpressing multidrug resistance associated protein,”
Eur. J. Cancer , 36 428
–434
(2000). Google Scholar
W. D. Stein
,
“Kinetics of the multidrug transporter (P-glycoprotein) and its reversal,”
Physiol. Rev. , 77 545
–590
(1997). Google Scholar
E. J. F. Demant
and
E. Friche
,
“Kinetics of anthracycline accumulation in multidrug-resistant tumor cells: relationship to drug lipophilicity and serum albumin binding,”
Biochem. Pharmacol. , 56 1209
–1217
(1998). Google Scholar
D. Kessel
,
“Exploring multidrug resistance using rhodamine 123,”
Cancer Commun. , 1 145
–149
(1989). Google Scholar
S. M. Doglia
,
L. Bianchi
,
R. Colombo
,
N. Allam
,
H. Morjani
,
M. Manfait
, and
A. M. Villa
,
“Confocal fluorescence microscopy of living cells,” 4th International Conference on Laser Applications in life Sciences, Laser Study of Macroscopic Biosystems,”
Proc. SPIE , 1922 126
–134
(1992). Google Scholar
J. M. Millot
,
S. Sharonov
, and
M. Manfait
,
“Scanning microspectro-fluorometry of rhodamine 123 in multidrug-resistant cells,”
Cytometry , 17 50
–58
(1994). Google Scholar
G. Arancia
,
A. Molinari
,
A. Calcabrini
,
G. Citro
,
A. M. Villa
,
A. Verdina
, and
G. Zupi
,
“Effects of sequential combinations of N-methylformamide with adriamycin on cultured melanoma cells (M14),”
Exp. Mol. Pathol. , 60 12
–26
(1994). Google Scholar
A. Calcabrini
,
A. M. Villa
,
A. Molinari
,
S. M. Doglia
, and
G. Arancia
,
“Influence of N-methylformamide on the intracellular transport of doxorubicin,”
Eur. J. Cell Biol. , 72 61
–69
(1997). Google Scholar
G. Arancia
,
A. Calcabrini
,
S. Meschini
, and
A. Molinari
,
“Intracellular distribution of anthracyclines in drug-resistant cells,”
Cytotechnology , 27 95
–111
(1998). Google Scholar
T. J. Preston
,
A. Abadi
,
L. Wilson
, and
G. Singh
,
“Mitochondrial contributions to cancer cell physiology, potential for drug development,”
Adv. Drug Delivery Rev. , 49 45
–61
(2001). Google Scholar
L. J. Robinson
,
W. K. Roberts
,
T. T. Ling
,
D. Lamming
,
S. S. Sternberg
, and
P. D. Roepe
,
“Human MDR 1 Protein Overexpression Delays the Apoptotic Cascade in Chinese Hamster Ovary Fibroblasts,”
Biochemistry , 36 11169
–11178
(1997). Google Scholar
M. J. Smyth
,
E. Krasovskis
,
V. R. Sutton
, and
R. W. Johnstone
,
“The drug efflux protein, P-glycoprotein, additionally protects drug-resistant tumor cells from multiple forms of caspase-dependent apoptosis,”
Proc. Natl. Acad. Sci. U.S.A. , 95 7024
–7029
(1998). Google Scholar
R. W. Johnstone
,
E. Cretney
, and
M. J. Smyth
,
“P-glycoprotein protects leukemia cells against caspase-dependent, but not caspase-independent, cell death,”
Blood , 93 1075
–1085
(1999). Google Scholar
P. Costantini
,
E. Jacotot
,
D. Decaudin
, and
G. Kroemer
,
“Mitochondrion as a novel target of anticancer chemotherapy,”
J. Natl. Cancer Inst. , 92 1042
–1053
(2000). Google Scholar
A. D. Schimmer
,
D. W. Hedley
,
L. Z. Penn
, and
D. Minden
,
“Receptor- and mitochondrial-mediated apoptosis in acute leukemia, a translational view,”
Blood , 98 3541
–3553
(2001). Google Scholar
K. Jung
and
R. Reszka
,
“Mitochondria as subcellular targets for clinically useful anthracyclines,”
Adv. Drug Delivery Rev. , 49 87
–105
(2001). Google Scholar
S. Desagher
and
J.-C. Martinou
,
“Mitochondria as the central control of apoptosis,”
Trends Cell Biol. , 10 369
–377
(2000). Google Scholar
G. Kroemer
and
J. C. Reed
,
“Mitochondrial control of cell death,”
Nat. Med. , 6 513
–519
(2000). Google Scholar
V. P. Skulachev
,
“The programmed death phenomena, aging, and the Samurai law of biology,”
Exp. Gerontol. , 36 995
–1024
(2001). Google Scholar
L. V. Johnson
,
M. L. Walsh
, and
L. B. Chen
,
“Localization of mitochondria in living cells with rhodamine 123,”
Proc. Natl. Acad. Sci. U.S.A. , 77 990
–994
(1980). Google Scholar
L. V. Johnson
,
M. L. Walsh
,
B. J. Bockus
, and
L. B. Chen
,
“Monitoring of relative mitochondrial membrane potential in living cells by fluorescence microscopy,”
J. Cell Biol. , 88 526
–535
(1981). Google Scholar
S. Davis
,
M. J. Weiss
,
J. R. Wong
,
T. J. Lampidis
, and
L. B. Chen
,
“Mitochondrial and plasma membrane potentials cause unusual accumulation and retention of rhodamine 123 by human breast adenocarcinoma-derived MCF-7 cells,”
J. Biol. Chem. , 260 13844
–13850
(1985). Google Scholar
T. Tsuruo
,
H. Iida
,
S. Tsukagoshi
, and
Y. Sakurai
,
“Overcoming of vincristine resistance in P388 leukemia in vivo and in vitro through enhanced cytotoxicity of vincristine and vinblastine by verapamil,”
Cancer Res. , 41 1967
–1972
(1981). Google Scholar
T. Tsuruo
,
H. Iida
,
M. Nojiri
,
S. Tsukagoshi
, and
Y. Sakurai
,
“Circumvention of vincristine and Adriamycin resistance in vitro and in vivo by calcium influx blockers,”
Cancer Res. , 43 2905
–2910
(1983). Google Scholar
A. Trussardi
,
G. Poitevin
,
M. C. Gorisse
,
M. J. Faroux
,
H. Bobichon
,
C. Delvincourt
, and
J. C. Jardiller
,
“Sequential overexpression of LRP and MRP but not P-gp 170 in VP16-selected A549 adenocarcinoma cells,”
Int. J. Oncol. , 13 543
–548
(1998). Google Scholar
V. P. Skulachev
,
“Mitochondrial filaments and clusters as intracellular power-transmitting cables,”
Trends Biochem. Sci. , 26 23
–29
(2001). Google Scholar
A. Molinari
,
M. Cianfriglia
,
S. Meschini
,
A. Calcabrini
, and
G. Arancia
,
“P-glycoprotein expression in the Golgi apparatus of multidrug-resistant cells,”
Int. J. Cancer , 59 789
–795
(1994). Google Scholar
N. G. Lipsky
and
R. E. Pagano
,
“A vital stain for the Golgi apparatus,”
Science , 28 745
–747
(1985). Google Scholar
M. Castedo
,
K. Ferri
,
T. Roumier
,
D. Me´tivier
,
N. Zamzami
, and
G. Kroemer
,
“Quantitation of mitochondrial alterations associated with apoptosis,”
J. Immunol. Methods , 265 39
–47
(2002). Google Scholar
J. Frederic
,
“Recherches Cytologiques sur le chondriome normal ou soumis a` l’expe´rimentation dans des cellules vivantes cultive´es in vitro,”
Arch. Biol. (Liege) , 69 167
–342
(1958). Google Scholar
T. Shimada
,
K. Horita
,
M. Murakami
, and
R. Ogura
,
“Morphological studies of different mitochondrial populations in monkey myocardial cells,”
Cell Tissue Res. , 238 577
–582
(1984). Google Scholar
V. N. Dedov
,
P. J. Armati
, and
B. D. Roufogalis
,
“Three-dimensional organisation of mitochondrial clusters in regenerating dorsal root ganglion (DRG) neurons from neonatal rats: evidence for mobile mitochondrial pools,”
J. Peripher Nerv. Syst. , 5 3
–10
(2000). Google Scholar
J. Bereiter-Hahn
,
“Behavior of mitochondria in the living cell,”
Int. Rev. Cytol. , 122 1
–63
(1990). Google Scholar
T. J. Collins
,
M. J. Berridge
,
P. Lipp
, and
M. D. Bootman
,
“Mitochondria are morphologically and functionally heterogeneous within cells,”
EMBO J. , 21 1616
–1627
(2002). Google Scholar
M. R. Duchen
,
“Contributions of mitochondria to animal physiology: from homeostatic sensor to calcium signalling and cell death,”
J. Physiol. (Lond.) , 516 1
–17
(1999). Google Scholar
R. Rizzuto
,
M. Brini
,
M. Murgia
, and
T. Pozzan
,
“Microdomains with high
Ca2+
close to IP3-sensitive channels that are sensed by neighboring mitochondria,”
Science , 262 744
–747
(1993). Google Scholar
G. Hajnoczky
,
G. Csordas
,
M. Madesh
, and
P. Pacher
,
“The machinery of local
Ca2+
signalling between sarco-endoplasmic reticulum and mitochondria,”
J. Physiol. (Lond.) , 529 69
–81
(2000). Google Scholar
G. Csordas
,
A. P. Thomas
, and
G. Hajnoczky
,
“Quasi-synaptic calcium signal transmission between endoplasmic reticulum and mitochondria,”
EMBO J. , 18 96
–108
(1999). Google Scholar
A. M. Lawrie
,
R. Rizzuto
,
T. Pozzan
, and
A. W. M. Simpson
,
“A role for calcium influx in the regulation of mitochondrial calcium in endothelial cells,”
J. Biol. Chem. , 271 10753
–10759
(1996). Google Scholar
M. K. Park
,
M. C. Ashby
,
G. Erdemli
,
O. H. Petersen
, and
A. V. Tepikin
,
“Perinuclear, perigranular and sub-plasmalemmal mitochondria have distinct functions in the regulation of cellular calcium transport,”
EMBO J. , 20 1863
–1874
(2001). Google Scholar
S. Kirischuk
,
J. Neuhaus
,
A. Verkhratsky
, and
H. Kettenmann
,
“Preferential localization of active mitochondria in process tips of immature retinal oligodendrocytes,”
NeuroReport , 6 737
–741
(1995). Google Scholar
R. S. Weinstein
,
S. M. Jakate
,
J. M. Dominguez
,
M. D. Lebovitz
,
G. K. Koukoulis
,
J. R. Kuszak
,
L. F. Klusens
,
T. M. Grogan
,
T. J. Saclarides
, and
I. B. Roninson
,
“Relationship of the expression of the multidrug resistance gene product (P-glycoprotein) in human colon carcinoma to local tumor aggressiveness and lymph node metastasis,”
Cancer Res. , 51 2720
–2726
(1991). Google Scholar
M. Hojo
,
T. Morimoto
,
M. Maluccio
,
T. Asano
,
K. Morimoto
,
M. Lagman
,
T. Shimbo
, and
M. Suthanthiran
,
“Cyclosporine induces cancer progression by a cell-autonomous mechanism,”
Nature (London) , 397 530
–534
(1999). Google Scholar
G. Amuthan
,
G. Biswas
,
S.-Y. Zhang
,
A. Klein-Szanto
,
C. Vijayasarathy
, and
N. G. Avadhani
,
“Mitochondria-to-nucleus stress signaling induces phenotypic changes, tumor progression and cell invasion,”
EMBO J. , 20 1910
–1920
(2001). Google Scholar
J. E. De Larco
,
B. R. K. Wuertz
,
J. C. Manivel
, and
L. T. Furcht
,
“Progression and enhancement of metastatic potential after exposure of tumor cells to chemotherapeutic agents,”
Cancer Res. , 61 2857
–2861
(2001). Google Scholar
E. F. Farias
,
J. A. Aguirre Ghiso
,
V. Ladeda
, and
E. Bal de Kier Joffe
,
“Verapamil inhibits tumor protease production, local invasion and metastasis development in murine carcinoma cells,”
Int. J. Cancer , 78 727
–734
(1998). Google Scholar
G. J. Randolph
,
S. Beaulieu
,
M. Pope
,
I. Sugawara
,
L. Hoffman
,
R. M. Steinman
, and
W. A. Muller
,
“A physiologic function for p-glycoprotein (MDR-1) during the migration of dendritic cells from skin via afferent lymphatic vessels,”
Proc. Natl. Acad. Sci. U.S.A. , 95 6924
–6929
(1998). Google Scholar
T. J. Collins
,
P. Lipp
,
M. J. Berridge
,
W. Li
, and
M. D. Bootman
,
“Inositol 1,4,5-trisphosphate-induced
Ca2+
release is inhibited by mitochondrial depolarization,”
Biochem. J. , 347 593
–600
(2000). Google Scholar
G. Szalai
,
R. Krishnamurthy
, and
G. Hajnoczky
,
“Apoptosis driven by IP(3)-linked mitochondrial calcium signals,”
EMBO J. , 18 6349
–61
(1999). Google Scholar
K. D. Bunting
,
“ABC transporters as phenotypic markers and functional regulators of stem cells,”
Stem Cells , 20 11
–20
(2002). Google Scholar
|
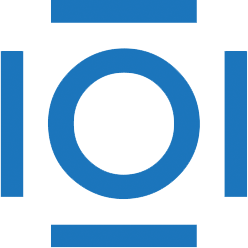
CITATIONS
Cited by 32 scholarly publications.
Luminescence
Resistance
Tumors
Confocal microscopy
Calcium
Image analysis
Optical filters